The Large Hadron Collider
Our understanding of the Universe is about to change...
The Large Hadron Collider (LHC) is a gigantic scientific instrument near Geneva, where it spans the border between Switzerland and France about 100 m underground. It is a particle accelerator used by physicists to study the smallest known particles – the fundamental building blocks of all things. It will revolutionise our understanding, from the minuscule world deep within atoms to the vastness of the Universe.
Two beams of subatomic particles called 'hadrons' – either protons or lead ions – will travel in opposite directions inside the circular accelerator, gaining energy with every lap. Physicists will use the LHC to recreate the conditions just after the Big Bang, by colliding the two beams head-on at very high energy. Teams of physicists from around the world will analyse the particles created in the collisions using special detectors in a number of experiments dedicated to the LHC.
There are many theories as to what will result from these collisions, but what's for sure is that a brave new world of physics will emerge from the new accelerator, as knowledge in particle physics goes on to describe the workings of the Universe. For decades, the Standard Model of particle physics has served physicists well as a means of understanding the fundamental laws of Nature, but it does not tell the whole story. Only experimental data using the higher energies reached by the LHC can push knowledge forward, challenging those who seek confirmation of established knowledge, and those who dare to dream beyond the paradigm.
How an accelerator works
Accelerators were invented to provide energetic particles to investigate the structure of the atomic nucleus. Since then, they have been used to investigate many aspects of particle physics. Their job is to speed up and increase the energy of a beam of particles by generating electric fields that accelerate the particles, and magnetic fields that steer and focus them.An accelerator comes either in the form of a ring (circular accelerator), where a beam of particles travels repeatedly round a loop, or in a straight line (linear accelerator), where the beam travels from one end to the other. A number of accelerators may be joined together in sequence to reach successively higher energies, as at the accelerator complex at CERN.
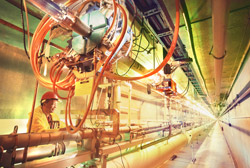
- Radiofrequency (RF) cavities and electric fields – these provide acceleration to a beam of particles. RF cavities are located intermittently along the beam pipe. Each time a beam passes the electric field in an RF cavity, some of the energy from the radio wave is transferred to the particles.
- Vacuum chamber – this is a metal pipe (also known as the beam pipe) inside which a beam of particles travels. It is kept at an ultrahigh vacuum to minimise the amount of gas present to avoid collisions between gas molecules and the particles in the beam.
- Magnets – various types of magnets are used to serve different functions. For example, dipole magnets are usually used to bend the path of a beam of particles that would otherwise travel in a straight line. The more energy a particle has, the greater the magnetic field needed to bend its path. Quadrupole magnets are used to focus a beam, gathering all the particles closer together (similar to the way that lenses are used to focus a beam of light).
An accelerator at home
A cathode ray tube (CRT) television set has the basic features of CERN’s accelerators. A filament inside the glass vacuum tube of the television set acts as a source of particles. When the filament is heated, electrons are set free by the increase in energy. The electrons are accelerated and guided through the vacuum of the CRT by an electromagnetic field, generated by a coil of wires. The television screen acts as a particle detector. As the high-energy electrons hit the back of the screen, they are detected and made visible in the colour pixels that make up the image.The LHC experiments
The six experiments at the LHC are all run by international collaborations, bringing together scientists from institutes all over the world. Each experiment is distinct, characterised by its unique particle detector.The two large experiments, ATLAS and CMS, are based on general-purpose detectors to analyse the myriad of particles produced by the collisions in the accelerator. They are designed to investigate the largest range of physics possible. Having two independently designed detectors is vital for cross-confirmation of any new discoveries made.
Two medium-size experiments, ALICE and LHCb, have specialised detectors for analysing the LHC collisions in relation to specific phenomena.
Two experiments, TOTEM and LHCf, are much smaller in size. They are designed to focus on ‘forward particles’ (protons or heavy ions). These are particles that just brush past each other as the beams collide, rather than meeting head-on
The ATLAS, CMS, ALICE and LHCb detectors are installed in four huge underground caverns located around the ring of the LHC. The detectors used by the TOTEM experiment are positioned near the CMS detector, whereas those used by LHCf are near the ATLAS detector.
The standard package
The theories and discoveries of thousands of physicists over the past century have resulted in a remarkable insight into the fundamental structure of matter: everything in the Universe is found to be made from twelve basic building blocks called fundamental particles, governed by four fundamental forces. Our best understanding of how these twelve particles and three of the forces are related to each other is encapsulated in the Standard Model of particles and forces. Developed in the early 1970s, it has successfully explained a host of experimental results and precisely predicted a wide variety of phenomena. Over time and through many experiments by many physicists, the Standard Model has become established as a well-tested physics theory.Matter particles
Everything around us is made of matter particles.These occur in two basic types called quarks and leptons.Each group consists of six particles, which are related in pairs, or ‘generations’. The lightest and most stable particles make up the first generation, whereas the heavier and less stable particles belong to the second and third generations. All stable matter in the Universe is made from particles that belong to the first generation; any heavier particles quickly decay to the next most stable level.
The six quarks are paired in the three generations – the 'up quark' and the 'down quark' form the first generation, followed by the 'charm quark' and 'strange quark', then the 'top quark' and 'bottom quark'. The six leptons are similarly arranged in three generations – the 'electron' and the 'electron-neutrino', the 'muon' and the 'muon-neutrino', and the 'tau' and the 'tau-neutrino'. The electron, the muon and the tau all have an electric charge and a mass, whereas the neutrinos are electrically neutral with very little mass.
Forces and carrier particles
There are four fundamental forces at work in the Universe: the strong force, the weak force, the electromagnetic force, and the gravitational force. They work over different ranges and have different strengths. Gravity is the weakest but it has an infinite range. The electromagnetic force also has infinite range but it is many times stronger than gravity. The weak and strong forces are effective only over a very short range and dominate only at the level of subatomic particles. Despite its name, the weak force is much stronger than gravity but it is indeed the weakest of the other three. The strong force is, as the name says, the strongest among all the four fundamental interactions.We know that three of the fundamental forces result from the exchange of force carrier particles, which belong to a broader group called ‘bosons’. Matter particles transfer discrete amounts of energy by exchanging bosons with each other. Each fundamental force has its own corresponding boson particle – the strong force is carried by the ‘gluon’, the electromagnetic force is carried by the ‘photon’, and the ‘W and Z bosons’ are responsible for the weak force. Although not yet found, the ‘graviton’ should be the corresponding force-carrying particle of gravity.
The Standard Model includes the electromagnetic, strong and weak forces and all their carrier particles, and explains extremely well how these forces act on all the matter particles. However, the most familiar force in our everyday lives, gravity, is not part of the Standard Model. In fact, fitting gravity comfortably into the framework has proved to be a difficult challenge. The quantum theory used to describe the micro world, and the general theory of relativity used to describe the macro world, are like two children who refuse to play nicely together. No one has managed to make the two mathematically compatible in the context of the Standard Model. But luckily for particle physics, when it comes to the minuscule scale of particles, the effect of gravity is so weak as to be negligible. Only when we have matter in bulk, such as in ourselves or in planets, does the effect of gravity dominate. So the Standard Model still works well despite its reluctant exclusion of one of the fundamental forces.
So far so good, but...
...it is not time for physicists to call it a day just yet. Even though the Standard Model is currently the best description we have of the subatomic world, it does not explain the complete picture. The theory incorporates only three out of the four fundamental forces, omitting gravity. Alas, Newton would be turning in his grave! There are also important questions it cannot answer, such as what is dark matter, what happened to the missing antimatter, and more.Last but not least, an essential ingredient of the Standard Model, a particle called the Higgs boson, has yet to be found in an experiment. The race is on to hunt for the Higgs – the key to the origin of particle mass. Finding it would be a big step for particle physics, although its discovery would not write the final ending to the story.
So despite the Standard Model's effectiveness at describing the phenomena within its domain, it is nevertheless incomplete. Perhaps it is only a part of a bigger picture that includes new physics that has so far been hidden deep in the subatomic world or in the dark recesses of the Universe. New information from experiments at the Large Hadron Collider are sure to help us find more of these missing pieces.
No comments:
Post a Comment